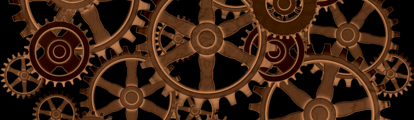
Refractive Surgery's Personalized Revolution
A better understanding of corneal biomechanics through computer modeling will transform the way we approach refractive surgery
Ophthalmologists have been leveraging the cornea’s biomechanical response for refractive purposes for over a century. The first reported case of astigmatic keratotomy dates back to 1885 (1), and keratorefractive surgery – as well as our understanding of how the cornea responds to it – has continued to develop ever since. Today, we have access to advanced imaging techniques that are able to measure the minute alterations in corneal shape that drive changes in the optical performance of the eye. Although most established forms of refractive surgery have satisfied rigorous safety and effectiveness criteria, there is an increasing desire among surgeons and patients to further optimize individual outcomes and postoperative stability. To meet this need, I believe we need a better working appreciation of corneal structural mechanics – not just an abstract intuition about mechanical responses (or weakly correlated predictors of those responses), but practical tools that can consolidate the complexities of a three-dimensional (3D) structural response problem into personalized guidance that can be used in our daily clinical practice.
Corneal biomechanics over the years
Arguably, surgeons had a greater appreciation for the importance of corneal biomechanics when refractive surgery was purely incisional. Not long after Tsutomu Sato began experimenting with anterior and posterior keratotomy for refractive correction and Svyatoslav Fyodorov refined radial keratotomy for myopia, ophthalmic surgeons were routinely exploiting their working knowledge of the biomechanics of the cornea to produce refractive change. But when laser ablation arose as the dominant mode of refractive correction in the 1990s, a narrower concept of refractive surgery as pure “shape subtraction” was adopted. That view neglected, or at least minimized, the contribution of the corneal biomechanical response to surgical outcomes.
As the 1990s progressed, awareness of the practical importance of biomechanics began to re-emerge. This was a period of rapid evolution of corneal imaging technology that saw more widespread use of Placido topography and introduction of new optical tomography devices. Several clinical phenomena suggested the continued relevance of biomechanical factors, even in laser refractive surgery, including: unexpected corneal flattening in phototherapeutic keratectomy (PTK) despite use of optically neutral ablation profiles; the need for empirical adjustment of the programmed laser treatment through surgeon nomograms; evidence of late post-radial keratotomy refractive drift; refractive regression after LASIK or PRK in some patients; and most importantly, postoperative corneal ectasia. These and other observations prompted a more nuanced understanding of the role of biomechanics: even in refractive procedures where biomechanical change is not the primary mechanism of action, it remains an important, often performance-limiting influence on treatment precision and stability.
The early 2000s saw the introduction of femtosecond lasers in refractive surgery. Far more impactful than just making “bladeless” LASIK a possibility, this technology supported unprecedented levels of treatment reproducibility in refractive surgery by enhancing the precision of the flap creation process and revitalizing incisional procedures by offering a highly customizable alternative to manual astigmatic keratotomy. At the same time, it ushered in a new class of intrastromal procedures such as small incision intrastromal lenticule extraction (SMILE) that made it possible to correct refractive error while deliberately sparing the corneal stroma’s most mechanically resilient anterior layers.
Building on their experimental work in the mid-1990s, Theo Seiler and his team reported the first clinical use of corneal cross-linking (CXL) in 2003. This was a momentous breakthrough in clinical ophthalmology and a major milestone in the timeline of corneal biomechanics: for the first time, a treatment that enhanced corneal biomechanical properties was used to treat keratoconus, a condition in which a deficit in corneal material properties is the final common pathway to progression. Just two years later, a commercially available tool for measuring corneal biomechanical properties (the Ocular Response Analyzer, Reichert Instruments) was introduced and broke through the first major barrier to understanding and using biomechanical measurement in clinical practice. Hundreds of studies have followed that have further established the role of this measurement method and its derivatives as independent predictors of keratoconus and Marfan syndrome, post-LASIK ectasia risk, and even progression of glaucomatous visual field and nerve fiber layer loss. More recently, the Corvis ST from Oculus was introduced, and it provides direct visualization and measurement of corneal deformation behavior in one corneal meridian, and major progress is now being made toward full spatial mapping of corneal elastic properties using technologies such as OCT elastography and Brillouin scattering microscopy. These newer technologies have the potential to detect localized abnormalities in biomechanical properties and produce a patient-specific 3D corneal biomechanical fingerprint. With further study, they will likely lead to breakthroughs in assessing ectasia susceptibility in refractive surgery candidates and establishing a prognosis in young keratoconus suspects facing decisions about early intervention with CXL.
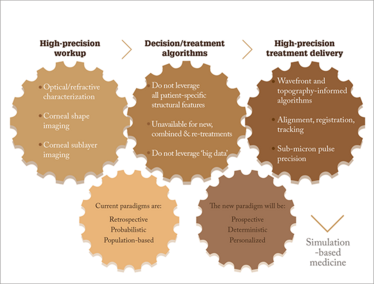
Figure 1. In refractive surgery, there is currently a precision gap between workup and treatment delivery. Computational tools that integrate large amounts of patient-specific data can aid with treatment decisions and help us move towards more personalized, simulation-based medicine.
Individualized treatment planning: The missing link
It’s clear that, remarkable advances have been made in measurement and treatment technologies in cornea and refractive surgery (Figure 1). We have very precise clinical tools to characterize the 3D anatomy of the eye and its optical performance. We have excimer and femtosecond laser systems with sub-micron pulse precision and tracking systems that offer exquisite opportunities for customizing treatments, and more sophisticated UV delivery systems for customized CXL are already available outside of the US. Devices for mapping the corneal biomechanical properties are around the corner. But when it comes to treatment planning, current paradigms have not kept pace with the dramatic growth in available information for preoperative planning or the precision of treatment systems. By leaving an enormous amount of patient data on the table, some of which is very likely to enhance the ability to predict an individual’s outcome, the precision of the entire treatment process is limited by the coarseness and low patient specificity of the planning tool.
The current degree of personalization varies greatly across procedures. In CXL for keratoconus, a standardized treatment is typically used for all candidate patients without modification. Most conventional excimer laser treatment algorithms and nomogram software make use of a very limited subset of data, namely the refractive sphere and cylinder and perhaps a corneal curvature value. Even though maps of corneal elevation, curvature and thickness are usually obtained preoperatively, this information is used only to determine candidacy for surgery and not as input for the treatment algorithm. Wavefront-guided and topography-guided ablation algorithms are exceptions, and by incorporating whole-eye aberrations or anterior corneal elevation data, these procedures provide a higher level of personalization than convention treatments. Even with over 90 percent of myopic LASIK patients achieving 20/20 or better vision, there is an opportunity to improve predictability, and results in hyperopic and higher astigmatic corrections are less predictable. Emerging treatments such as crosslinking for lower refractive errors may not have obvious treatment algorithms to take into clinical trials, and nomograms for intracorneal ring segments and astigmatic keratotomy are more qualitative with less predictable outcomes. Algorithm modifications for combined treatments (for example, PRK and CXL), enhancement procedures, and treatments in atypical corneas (like relaxing incisions after keratoplasty) are either absent or only minimally patient-specific. Even though all of these procedures involve biomechanical interactions with the cornea, we don’t have a unifying method for planning that is grounded in structural principles.
Closing the precision gap with simulation-based planning
Current paradigms for optimizing outcomes in our patients are retrospective, probabilistic, and population-based. Nomograms are based on historical outcomes, and de novo outcomes are predicted by calculating the average historical response of a minimalistic representation of the new patient. Such history-based nomograms are helpful, and when they are specific to the surgeon, can capture environmental variables that influence outcomes. But thanks to computational advances and the increasing availability of high-resolution patient data, we are moving toward a more prospective, structurally deterministic, and personalized approach through simulation-based medicine.
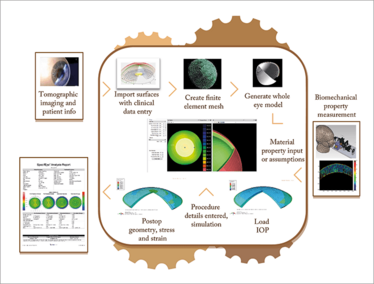
Figure 2. Workflow for a software-based treatment planning tool. Patient-specific data such as tomography, IOP and eventually biomechanical properties are imported, and the user enters the anticipated surgical parameters. Reports are generated showing the predicted outcome based on simulation and other machine learning strategies. The box includes the personal computer software processes and web-based finite element solver.
The relationship between corneal shape and visual performance is one of nature’s finest examples of a structure-function relationship, and presents an enormous opportunity for ophthalmology to lead in the area of simulation-based medicine. Investigation of computational biomechanical models as tools for predicting refractive surgery responses dates back to at least 1989. Our group and others have escalated efforts to apply such models to clinical prediction, and with proper validation, I believe they will change how we approach corneal and refractive surgery. It was computational modeling that demonstrated how strain redistribution leads to localized flattening in CXL, that tested hypotheses of keratoconus progression, and that provided a virtual trial for showing the potential of customized CXL patterns to maximize topographic normalization in keratoconus and correct myopia, hyperopia, and astigmatism without weakening the cornea. Studies examining the performance of models for predicting outcomes in LASIK and assessing biomechanical risk of ectasia are underway. Modeling has the makings of a universal planning tool, one that could dramatically increase the utility of biomechanical measurements by leveraging them in simulations.
The greatest advantage of modeling is that it provides a mechanism for combining nearly everything we know about and can measure from the patient’s eye and the proposed treatment. It then allows the user to subject the virtual version of that eye to that treatment, observe the predicted outcome, and consider the results during the planning of the actual treatment.
gives an overview of the workflow that we are developing to automate the process, and Figure 3 offers an example of a modeling result comparing the clinical and model-predicted axial curvature maps of a myopic crosslinking procedure from a collaboration between our laboratory, Avedro, and Burkhard Dick’s group in Bochum, Germany. In addition to importing patient data such as corneal geometry, axial eye length, clinical refraction, and eventually biomechanical properties, the model allows detailed specification of all surgical parameters and performs ray-tracing on pre- and post-treatment surfaces to estimate refractive change and higher order aberrations. Aside from its potential as a surgical guidance tool, modeling is currently being used to test hypotheses, explore novel treatment designs, refine treatment algorithms in preclinical simulations, and perform stress and strain-based risk assessments – all on a personal computer.
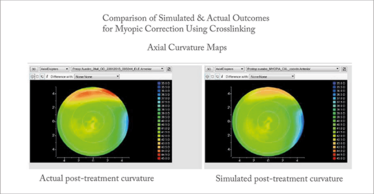
Figure 3. Example comparing the clinical and model-predicted axial curvature maps of a myopic crosslinking procedure. The predicted change in central curvature was -0.99D and actual change was -0.90D.
‘Big data’ will support even better prediction
The vision of the future of surgical planning that I’ve presented here focuses on ‘big data’ in the context of the individual: ensuring that the predictive model knows as much as possible about the eye and the treatment for the most effective “n of one” study possible. I have highlighted how this approach differs from the current approach of ‘customizing’ treatment based on historical outcomes for the average eye using regression equations that are agnostic to most of the available data and that do not explicitly account for the important structural effects we reviewed early in this article. The current approach is useful for reducing systematic error in prediction as long as the right information is included in the statistical analysis. But our knowledge of what is important to include is imperfect, especially for newer treatments and atypical eyes, and nomograms can only account for inter-individual differences in outcomes if they capture the personal factors that drive those differences.
However, this does not discount the value of big data beyond the individual eye. The rich datasets that are collected through the modeling process and the predictions that are generated across many simulations for many eyes and many procedures are an important source of model refinement and continuous improvement. Aggregating these results and comparing to actual outcomes data will allow automated learning mechanisms to provide “smarter” nomogram suggestions that incorporate structural predictions along with empirical performance information.
Imagine the refractive practice of five years’ time – one where the “precision gap” has been bridged by refined treatment planning technologies and greater levels of personalization. I believe that in that timespan, we will also incorporate corneal biomechanical measurements into our workflow. These tools will support increasingly more accurate predictions of the outcomes of procedures, and help identify which procedures and which treatment parameters will generate the most optimal outcome possible for each patient. We will be able to more objectively screen patients for refractive procedures using structural information in a data-driven, computationally-assisted process, one that helps us rule out eyes that aren’t safe for surgery and proceed with greater confidence in ambiguous cases.
A Concise Clinical Timeline Of Corneal Biomechanics
1960s
Biomechanics leveraged widely to produce incision-mediated refractive change
1980s
Photoablative refractive surgery is introduced and conceptualization of surgery as “shape-subtraction” undermines appreciation of importance of biomechanics
Late 1980s
First published finite element model of corneal refractive surgery
1990s
More widespread use of high-precision corneal imaging technology. Biomechanics in photoablative surgery increasingly recognized as an important source of outcome variability and instability (fluctuation, regression, ectasia)
2000s
Introduction of femtosecond laser for precision creation of flaps, incisions and eventually intrastromal lenticules and pockets
2003
Demonstrated potential of crosslinking to stabilize progressive biomechanical disease
2005
First clinical instrument commercialized to measure corneal biomechanics
2010s
Computational modeling used to explain flattening effect of crosslinking, test hypotheses of keratoconus progression, and show potential for using personalized crosslinking patterns to maximize topographic normalization in keratoconus and produce refractive effects – without weakening the cornea
Today
Accelerated development of tools for mapping corneal biomechanical properties and software for simulation-based treatment planning
Today’s surgical outcomes are excellent. But with better utilization of rich patient-specific datasets and computer modeling of structural mechanics, we can narrow the precision gap in refractive surgery planning. As a result, tomorrow’s surgery will be safer, more predictable, and characterized by even better outcomes.
William J. Dupps Jr. is a cornea and refractive surgeon at the Cole Eye Institute with appointments in Ophthalmology, Biomedical Engineering and Transplant at Cleveland Clinic, and an Adjunct Associate Professor of Biomedical Engineering at Case Western Reserve University in Cleveland, Ohio. He is founder of OptoQuest Inc., a Cleveland Clinic company focused on commercial translation of biomechanical measurement technology and a clinical modeling tool, SpecifEye™.
- LJ Schiotz, “Hin Fall von hochgradigem Horn-hautstastig-ma-tis-mus nach Staarextraction: Bessergung auf operativem Wege”, Arch Augenheilkd, 15, 178, (1885).
William J. (BJ) Dupps Jr. is a cornea and refractive surgeon at the Cole Eye Institute with appointments in Ophthalmology, Biomedical Engineering and Transplant at Cleveland Clinic, and an Adjunct Associate Professor of Biomedical Engineering at Case Western Reserve University in Cleveland, Ohio. He is founder of OptoQuest Inc., a Cleveland Clinic company focused on commercial translation of biomechanical measurement technology and a clinical modeling tool, SpecifEye™.