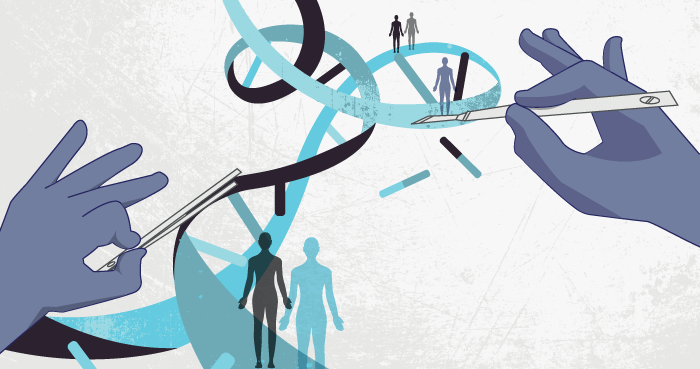
Approximately 1 in 2,000 people worldwide are affected by inherited retinopathies (1) but few treatment options are available for retinal degeneration. There is also no cure for glaucoma, which affects 60 million people worldwide (2) and is caused by degeneration of retinal ganglion cells (RGCs) and their axon bundles that form the optic nerve. The FDA’s 2017 approval of LUXTURNA to treat inherited retinal degeneration caused by biallelic mutations in RPE65 has established viral vectors as a viable clinical therapy to monogenic retinal disease. Furthermore, advances in pluripotent stem cell techniques have enabled retinal pigment epithelium (RPE) to reach clinical trials as a cell therapy for treating age-related macular degeneration (AMD) (3). Now it is time to talk about in vivo reprogramming: a novel therapeutic approach that will circumvent the issues with subretinal transplantation of cell therapies and the challenge of targeting every monogenic condition with gene therapy.
A refresher in how the eye works…
To enable vision, photoreceptors in the neural retina absorb photons and cascade a signal through isomerization of 11-cis retinal and conformational changes in membrane-bound opsin proteins. This phototransduction causes hyperpolarization and closure of cGMP-gated cation channels, and the signal is propagated to bipolar, horizontal, then RGCs in the retina. The photoreceptor outer segments, where opsins are densely populated, are phagocytosed by the underlying RPE. The RPE monolayer forms a blood-retina barrier, which means there is an immune privilege for retinal cell therapies. Typically, RGCs are responsible for transmitting the visual information from photoreceptors to the brain; however, a sub-population of RGCs have been shown to be photosensitive and involved in the regulation of circadian rhythms. The axons of RGCs form the optic nerve which is responsible for transmitting visual information from the retina to the occipital lobe.
Out of the two photoreceptor types, rods represent up to 95 percent of the photoreceptor population and are responsible for low light and peripheral vision, while cones occupy only 5 percent of the total photoreceptor population and enable central vision and color perception (4). Rods express rhodopsin protein, whereas cones express S/M/L opsins and are primarily found in the fovea for central visual acuity. NRL is a transcription factor that defines rod cell identity, whereas CRX is highly expressed in both rods and cones (5). MITF is well-established as a transcription factor found predominantly expressed in RPE (6), whereas RGCs express ATOH7 and BRN3a/BRN3b transcription factors (7). By harnessing the power of transcription factors to control retinal cell identity and trans-differentiation, data-driven cell conversions can lead to the development of new cell therapies and in vivo reprogramming approaches to treat retinal degeneration.
Degeneration of the retina and the optic nerve
Let’s go through different types of retina and optic nerve degeneration and discuss what place in vivo reprogramming may have in addressing them.
AMD is the most common form of retinal degeneration and affects up to 170 million people worldwide (9). There are many causes and no approved treatments that can reverse the degeneration of both RPE and photoreceptor layers. Treatments for wet AMD include anti-VEGF therapy that prevent the formation of new blood vessels. Human embryonic stem cell (ESC)-derived RPE has been in phase I/II clinical trials to evaluate safety and efficacy (9); however, there remains no approved cell or gene therapy to prevent, and potentially reverse, the progression of AMD.
Inherited retinal degenerations are heterogenous diseases that result in a progressive loss of photoreceptors and visual acuity. Retinitis pigmentosa (RP) is a group of phenotypically and genetically variable inherited retinal disorders that affect up to 1 in 4,000 people in the US and worldwide (10). RP is typically defined by mutations in key photoreceptor genes, with over 50 genes known to be affected (10), including rhodopsin, RPE65, USH2A, PDE6A, and PDE6B. Mutations in rhodopsin are the most prevalent cause (accounting for under 18 percent) of autosomal dominant RP (11). Less commonly, RP can be autosomal recessive or X-linked recessive, as in the case of RP2. Cone-rod dystrophies are estimated to affect 1 in 30,000–40,000 people and ABCA4 mutations are responsible for 30–60 percent of autosomal recessive cases. Meanwhile, mutations in GUCY2D and CRX genes are responsible for up to half of the cases of autosomal dominant cone-rod dystrophies (12). Gene therapies have already proven successful in treating monogenic RP and there is substantial commercial interest in retinal gene therapy, while cell therapies also have the potential to treat all RP subtypes. However, in vivo reprogramming has the potential to be a more efficacious approach to late-stage retinal degeneration as photoreceptor integration and synaptic connections are more likely than with cell transplantation. This is because it takes advantage of Müller glia in situ; they are proximal to photoreceptors and have existing gap junctions with retinal neurons.
Glaucoma involves degeneration of the optic nerve that connects the retina to the brain and accounts for 12 percent of global blindness, making it the second most common cause of blindness worldwide (13). It is characterized by RGC death – most often caused by elevated intraocular pressure that results in deformation of the lamina cribrosa pores located at the optic nerve head. The lamina cribrosa is a porous extra-cellular matrix where the RGCs exit the retina; their axons form the optic nerve (14). Mutations in the MYOC gene are the most common genetic causes of primary open-angle glaucoma (15). Current treatments focus on lowering of intraocular pressure to slow progression of glaucoma; however, cell and gene therapies offer the greatest hope for reversing the damage to vision caused by glaucoma.
Cell therapies for retinal degeneration
Why has the eye proved to be well-suited for cell and gene therapies? It is easily accessible, immune-privileged, and well suited for clinical imaging because of the transparent cornea and lens. For example, the retina can be clinically monitored using imaging techniques such as optical coherence tomography and fluorescence adaptive optics scanning light ophthalmoscopy imaging (16). In this way, engrafted cells and retinal structure can be monitored after therapy, without termination of the study. Therefore, the safety and efficacy of cell and gene therapies can be readily assessed over time in animals and human clinical studies.
ESCs and induced pluripotent stem cells (iPSCs) are clinically valuable as a source of retinal cell types for research and application. Retinal organoids generated from these cells have helped to significantly advance the understanding of retina development (17, 18), and they have also provided an effective tool to study photoreceptor isolation and transplantation (19). There have been several clinical trials to test the safety and efficacy of RPE derived from ESCs/iPSCs for the treatment of AMD (3). RPE can be spontaneously produced from iPSCs by removal of FGF2 from pluripotency media, or more efficiently generated by the addition of small molecules such as nicotinamide and activin A (20, 21). The delivery of RPE can be as a cell suspension or as a cell sheet through subretinal implantation. Several studies have also tried to transdifferentiate fibroblasts to RPE for clinical transplantation, using transcription factors including MITF (22, 23). Clinical trials have reached phase II for RPE transplantation to treat AMD and several strategies are being developed to more effectively deliver RPE generated from pluripotent stem cells.
Cell therapies are an attractive option for treating inherited retinal disorders as they have the potential to treat all variants of RP mutations by regenerating photoreceptors. Importantly, allogeneic therapies would not carry the host mutation and there is a blood-retina barrier formed by the RPE that grants immune privilege. Recent efforts have focused on generating Müller glia that can be isolated from organoids for sub-retinal transplantation and improvement of RGC function (24), while cone photoreceptor density can be increased in organoids so they can be isolated for transplantation (25). Fetal retinal progenitor cells (RPCs) are currently in phase I/IIa clinical trials (26), and have shown promising results in a phase IIb clinical trial for the treatment of RP (27). The use of RPCs as a cell therapy continues to be an exciting prospect for the treatment of inherited retinopathies.
There are several published protocols for the generation of RGCs from iPSCs and retinal organoids (28), and they arise early in retinal development. Their application as a cell therapy remains difficult because of the axonal length required to connect the retina and visual cortex in the posterior region of the brain. For RGC cell therapies to be successful, the elevated intraocular pressure and lamina cribrosa defects also need correcting to prevent further damage to the donor RGCs. Though RGC therapy is challenging, it offers a very real prospect of optic nerve regeneration as there are few options for gene therapy.
Gene therapies for retinal degeneration
Gene therapy strategies aim to silence, replace, or repair defective genes that form the basis of inherited retinal diseases, or they may target pathways involved in inflammation or visual transduction. Adeno-associated viruses (AAVs) are non-pathogenic and have been shown to effectively target retinal cells, such as photoreceptors and RPE, after subretinal injection. The FDA recently approved LUXTURNA for the treatment of inherited retinal disorders using AAV vectors (29). Specifically, it is used for the treatment of Leber’s congenital amaurosis (LCA) or RP caused by confirmed biallelic RPE65 mutations. The approval has led to great interest in the development of AAV gene therapies for inherited retinal disorders.
The blood-retina barrier formed by the retinal pigment epithelium helps to prevent viral vector diffusion into other organs and eliciting an immune response. However, AAVs are limited in the size of cargo that they can deliver. Two examples of genes that are too big to package into AAVs are MY07A, related to Usher Syndrome, and ABCA4, which is mutated in Stargardt’s disease. These genes have been delivered via lentivirus to test their safety and efficacy (30, 31). A dual vector approach has recently been used to restore the function of ABCA4 in homozygous null mice, which is a novel way to increase AAV vector capacity, but the level of expression may not be enough to elicit a therapeutic effect (32). Other strategies to deliver gene therapies include using antisense oligonucleotides for transient expression, which have been used for CEP290 in LCA and QR-421a for LCA involving USH2A (33).
Retinal organoids have recently been used to model X-linked RP caused by RP2 mutations. Gene therapy delivered by AAVs can correct photoreceptor degeneration and thinning of the outer nuclear layer in RP2-mutated organoids from patients (34). RPGR has also been targeted by AAVs for a gene therapy approach to treating X-linked RP (35). To protect cone photoreceptors in a mouse model of retinal degeneration, microglia have been stimulated with TGF-β1 using AAVs. In three mouse models of RP carrying different mutations, AAV-mediated delivery of TGF-β1 rescued degenerating cones. The results suggest that TGF-β1 can protect vision and may benefit patients with neurodegeneration (36).
A novel gene therapy approach to AMD blocks inflammation caused by the complement system. Here, AAVs are used to deliver a proprietary protein after vitrectomy; phase I/II trials commenced in January 2019 to test the safety and efficacy of this gene therapy in humans for treating dry AMD, with no safety concerns to date (37). There is also evidence that gene therapies may also help glaucoma patients by targeting aquaporin-1 in the ciliary body using CRISPR-Cas9 to reduce intraocular pressure (38). Other research highlights a CRISPR-Cas9 approach to gene editing MYOC that has been able to lower intraocular pressure in glaucomatous mouse eyes (15). Cell and gene therapies have the potential to restore vision after retinal degeneration; however, the delivery and integration of these approaches remains problematic and the number of approved advanced therapeutic medicinal products is few. Here, we evaluate in vivo reprogramming of Müller glia as an innovative approach to treating late-stage retinal degeneration.
How does our platform work?
The MOGRIFY technology (42) was developed as a systematic means of identifying the key regulatory switches, such as an optimal combination of transcription factors, required to drive cell identity. The platform can be used to enhance existing stem-cell forward reprogramming methods or can bypass development pathways altogether, affecting a direct trans-differentiation between a mature cell type to another mature cell type.
The platform follows a step-wise scientific approach to driving cell identity. Firstly, the RNA of the source and target cell types is sequenced in order to compare the gene expression levels in the context of all other possible cell types.
Simultaneously, local transcriptomic regulatory networks are built around each regulatory gene in the human genome to calculate and rank the effect of the genes on the desired cell conversion by querying large-scale regulatory networks. The optimal combination of key regulators is predicted to maximize network coverage while avoiding redundancies.
Once this optimal combination has been identified, the DNA sequence of each regulatory gene is encoded into delivery vectors, which are transduced into the source cell type causing changes in DNA expression profile and therefore switching the genetic programs of the cell which induces the conversion between source and target cell type.
In addition to identifying transcription factor-driven cell conversions, small molecules that are able to affect the expression of the key predicted transcription factors can be identified to create a small molecule conversion cocktail. This approach has the added benefit of not requiring the transduction of transcription factors and consequently holds greater potential as an in vivo reprogramming therapy.
Click here to watch our video
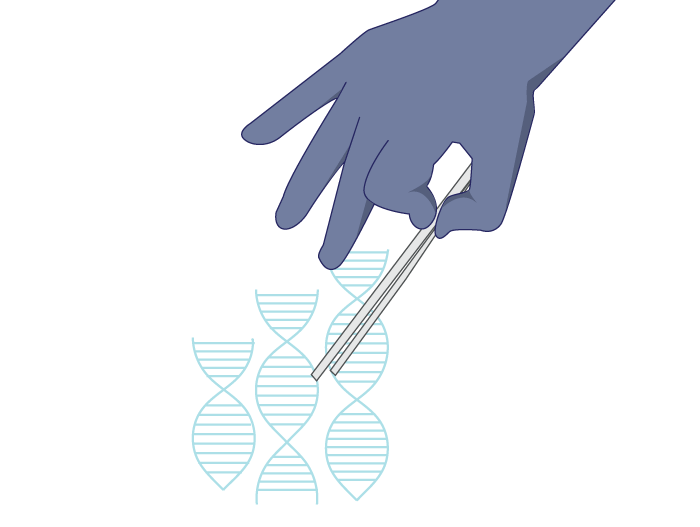
In vivo reprogramming for retinal regeneration
Since the discovery that OCT4, KLF4, SOX2, and cMYC transcription factors can reprogram somatic cells into induced pluripotent stem cells (39), there has been great interest in direct reprogramming for the development of new cell therapies. Examples of direct reprogramming include fibroblast conversion to neurons (40) and cardiomyocytes (41); however, it is difficult to predict the required reprogramming factors for cell conversion. Big-data approaches, such as the algorithm we developed (42), predict the transcription factors required to convert any cell type using RNA sequencing. Transcription factors are then ranked according to their influence over the target cells’ gene expression profile, and the optimal transcription factors are then used to convert cell types in vitro and in vivo. There is precedent for reprogramming of retinal cell types, such as fibroblasts to rods (43) and in vivo reprogramming of Müller glia to rods (44). Thus, the application of transcription factors directly for in vivo reprogramming of retinal cell types is an exciting new therapeutic approach to photoreceptor degeneration.
Müller glia represent the best potential source in the retina for in vivo conversion to photoreceptors as they are the primary support cell of the retina and activate on injury. In the zebrafish retina, Müller glia cells have been shown to regenerate other retinal cell types after injury and this finding suggests they have a progenitor capacity (45). The ASCL1 gene was shown to be up-regulated in Müller glia that contributed to the regeneration of neurons. In rodents, Müller glia are not associated with retinal regeneration (suggested to be a result of epigenetic repression), but they have been stimulated to generate neurons by ASCL1 overexpression and use of a histone deacetylase inhibitor trichostatin A (46). Another method to reprogram Müller glia is by cell fusion with hematopoietic progenitors, which has been shown to stimulate their conversion to photoreceptor precursors (47). More recently, the transcription factors OTX2, CRX, and NRL have been used to reprogram Müller glia to rod photoreceptors in Gnat1rd17Gnat2cpfl3 double mutant mice (44). This study used beta-catenin to drive proliferation of Müller glia before subsequent reprogramming to rod photoreceptors in the mice that lack functional photoreceptors. Overall, there is significant promise in the potential of converting Müller glia to photoreceptors to reverse retinal degeneration.
RPE has been shown to have stem cell properties in vitro and is another candidate to regenerate photoreceptors, as it is adjacently located to them in the outer nuclear layer (48). However, RPE is a quiescent monolayer, and cell conversions of RPE to photoreceptors may be counterintuitive given its fundamental role in photoreceptor homeostasis.
In vivo reprogramming is also attractive for the regeneration of RGCs and the treatment of glaucoma. One strategy has been developed to convert amacrine interneurons into RGCs by overexpressing Atoh7, Brn3B, Sox4, Sox11, and Isl1, which are established transcription factors in RGC development (49). There is also evidence that Ascl1, Brn3b, and Isl1 transcription factors can convert mouse fibroblasts into induced RGCs (50). Overexpression of KLF4 has also been shown to induce the regeneration of RGCs in vivo, even though it is not essential for their development (51).
By using a systematic data-driven approach, we will be able to predict optimal transcription factor sets for the conversion of retinal cell types, to generate cells for therapy, and as a gene therapy for in vivo reprogramming.
Who is behind Mogrify?
The emergence of Mogrify as a start-up with commercial aspirations follows years of scientific work. As there are around 2,000 transcription factors and 400 human cell types, the science of direct cell reprogramming is likely to advance slowly if it relies on educated guesses of the best combinations of conversion drivers.
Based in Cambridge, UK, Mogrify wants to make the identification of the transcriptomic and epigenetic switches driving cell identity and cell maintenance more scalable and efficient using a computational framework that makes predictions based on next-generation sequencing, gene regulatory and epigenetic network information. In 2016, Mogrify co-founder Julian Gough – Programme Leader at the MRC Laboratory of Molecular Biology in Cambridge – and his collaborators discussed their progress toward that goal in a paper detailing the ability of their framework to correctly predict the transcription factors that facilitate two then-novel trans-differentiation mechanisms (42).
Back then, Mogrify had applied the framework to 173 cell types and 134 tissues and validated it by correctly predicting known and novel transdifferentiation routes. In 2019 and 2020, Mogrify secured funding and multiple awards, including Innovation Award at PharmaIntelligence Scrip Awards, and the AstraZeneca Life Science Innovation Award from the Business Weekly Awards. This year Julian Gough received the Cambridge Enterprise Academic Entrepreneur of the Year award in recognition of his work as a life science innovator and founder of Mogrify.
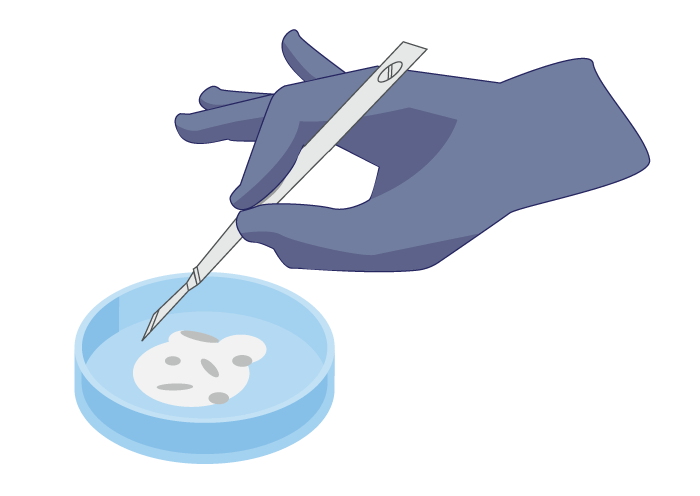
References
- MM Sohocki et al., “Prevalence of mutations causing retinitis pigmentosa and other inherited retinopathies,” Hum Mutat, 17, 42 (2001). PMID: 11139241.
- HA Quigley, “Glaucoma,” Lancet, 377, 1367 (2011). PMID: 21453963.
- M Mandai et al., “Autologous induced stem-cell-derived retinal cells for macular degeneration,” N Eng J Med, 376, 1038 (2017). PMID: 28296613.
- RS Molday, OL Moritz, “Photoreceptors at a glance,” J Cell Sci, 128, 4039 (2015). PMID: 26574505.
- PA Ruzycki et al., “CRX directs photoreceptor differentiation by accelerating chromatin remodeling at specific target sites,” Epigenetics Chromatin, 11, 42 (2018). PMID: 30068366.
- X Ma et al., “The transcription factor MITF in RPE function and dysfunction,” Prog Retin Eye Res, 73 (2019). PMID: 31242455.
- H Riazifar et al., “Chemically induced specification of retinal ganglion cells from human embryonic and induced pluripotent stem cells,” Stem Cell Transl Med, 3, 424 (2014). PMID: 24493857.
- KL Pennington, MM DeAngelis, “Epidemiology of age-related macular degeneration (AMD): associations with cardiovascular disease phenotypes and lipid factors,” Eye Vis (Lond), 3, 34 (2016). PMID: 28032115.
- TG Qiu, “Transplantation of human embryonic stem cell-derived retinal pigment epithelial cells (MA09-hRPE) in macular degeneration,” NPJ Regen Med, 4, 19 (2019). PMID; 31482011.
- National Eye Institute. "Retinitis Pigmentosa" (2019). Available at: https://bit.ly/371WAVZ.
- TP Dryja et al., “Mutations within the rhodopsin gene in patients with autosomal dominant retinitis pigmentosa,” N Engl J Med, 323, 1302 (1990). PMID: 2215617.
- Medline Plus, “Cone-rod dystrophy” (2020). Available at: https://bit.ly/2J26U8v.
- WHO, “Priority eye diseases” (2020). Available at: https://bit.ly/2J2HxDp.
- SC Park et al., “Lamina cribrosa depth in different stages of glaucoma,” Invest Ophthalmol Vis Sci, 56, 2059 (2015). PMID: 25722212.
- A Jain et al., “CRISPR-Cas9–based treatment of myocilin-associated glaucoma,” Proc Natl Acad Sci USA, 114, 11199 (2017). PMID: 28973933.
- E Aboualizadeh et al., “Imaging transplanted photoreceptors in living nonhuman primates with single-cell resolution,” Stem Cell Reports, 15, 482 (2020). PMID: 32707075.
- M Eiraku et al., “Self-organizing optic-cup morphogenesis in three-dimensional culture,” Nature, 472, 51 (2011). PMID: 21475194.
- F Regent et al., “A simple and efficient method for generating human retinal organoids,” Mol Vis, 26, 97 (2020). PMID: 32174751.
- J Lakowski et al., “Isolation of human photoreceptor precursors via a cell surface marker panel from stem cell-derived retinal organoids and fetal retinae,” Stem Cells, 36, 709 (2018). PMID: 29327488.
- H Hongisto et al., “Xeno- and feeder-free differentiation of human pluripotent stem cells to two distinct ocular epithelial cell types using simple modifications of one method,” Stem Cell Res Ther, 8, 291 (2017). PMID: 29284513.
- F Michelet et al., “Rapid generation of purified human RPE from pluripotent stem cells using 2D cultures and lipoprotein uptake-based sorting,” Stem Cell Res Ther, 11, 47 (2020). PMID: 32014053.
- IN Woogeng et al., “Inducing human retinal pigment epithelium-like cells from somatic tissue,” bioRxiv [Preprint] (2020). DOI: 10.1101/2020.07.27.215103.
- K Zhang et al., “Direct conversion of human fibroblasts into retinal pigment epithelium-like cells by defined factors,” 5, 48 (2014). PMID: 24474194.
- K Eastlake et al., “Phenotypic and functional characterization of Müller glia isolated from induced pluripotent stem cell-derived retinal organoids: improvement of retinal ganglion cell function upon transplantation,” Stem Cells Transl Med, 8, 775 (2019). PMID: 31037833.
- K Kruczek et al., “Differentiation and transplantation of embryonic stem cell-derived cone photoreceptors into a mouse model of end-stage retinal degeneration,” Stem Cell Reports, 8, 1659 (2017). PMID: 28552606.
- ReNeuron, “Phase I/IIa clinical trial in retinitis pigmentosa” (2020). Available at: https://bit.ly/3m7e61C.
- jCyte, “jCyte Inc. announces promising phase 2b results of jCell therapy in retinitis pigmentosa” (2020). Available at: https://bit.ly/2UTxJ0Y.
- S-L Ji, S-B Tang, “Differentiation of retinal ganglion cells from induced pluripotent stem cells: a review,” Int J Ophthalmol, 12, 152 (2019). PMID: 30662854.
- S Russell et al., “Efficacy and safety of voretigene neparvovec (AAV2-hRPE65v2) in patients with RPE65-mediated inherited retinal dystrophy: a randomised, controlled, open-label, phase 3 trial,” Lancet, 390, 849 (2017). PMID: 28712537.
- M Zallocchi et al., “EIAV-based retinal gene therapy in the shaker1 mouse model for usher syndrome type 1B: development of UshStat,” PLoS One, 9 (2014). PMID: 24705452.
- J Kong et al., “Correction of the disease phenotype in the mouse model of Stargardt disease by lentiviral gene therapy,” Gene Ther, 15, 1311 (2008). PMID: 18463687.
- ME McClements et al., “An AAV dual vector strategy ameliorates the stargardt phenotype in adult Abca4(-/-) Mice,” Hum Gen Ther, 30, 590 (2019)/ PMID: 30381971.
- I Vázquez-Domínguez et al., “Molecular therapies for inherited retinal diseases-current standing, opportunities and challenges,” Genes (Basel), 10, 654 (2019). PMID: 31466352.
- A Lane et al., “Modeling and rescue of RP2 retinitis pigmentosa using ipsc-derived retinal organoids,” Stem Cell Reports, 15, 67 (2020). PMID: 32531192.
- J Cehajic-Kapetanovic et al., “Initial results from a first-in-human gene therapy trial on X-linked retinitis pigmentosa caused by mutations in RPGR,” Nat Med, 26, 354 (2020). PMID: 32094925.
- SK Wang et al., “Microglia modulation by TGF-β1 protects cones in mouse models of retinal degeneration,” J Clin Invest, 130, 4360 (2020). PMID: 32352930.
- S Ellis et al., “GT005, a gene therapy for the treatment of dry age-related macular degeneration (AMD),” IOVS, 61, 2295 (2020).
- J Wu et al., “Gene therapy for glaucoma by ciliary body aquaporin 1 disruption using CRISPR-Cas9,” Mol Ther, 28, 820 (2020). PMID: 31981492.
- K Takahashi et al., “Induction of Pluripotent Stem Cells from Adult Human Fibroblasts by Defined Factors,” Cell, 131, 861 (2007). PMID: 18035408.
- Y Yang et al., “Rapid and efficient conversion of human fibroblasts into functional neurons by small molecules,” Stem Cell Reports, 13, 862 (2019). PMID: 31631018.
- Y Chen et al., “Direct reprogramming of fibroblasts into cardiomyocytes,” Stem Cell Res Ther, 8, 118 (2017). PMID: 28545505.
- OJL Rackham et al., “A predictive computational framework for direct reprogramming between human cell types,” Nat Genet, 48, 331 (2016). PMID: 26780608.
- B Mahato et al., “Pharmacologic fibroblast reprogramming into photoreceptors restores vision,” Nature, 581, 83 (2020). PMID: 32376950.
- K Yao et al., “Restoration of vision after de novo genesis of rod photoreceptors in mammalian retinas,” Nature, 560, 484 (2018). PMID: 30111842.
- J Wan, D Goldman, “Retina regeneration in zebrafish,” Curr Opin Genet Dev, 40, 41 (2016). PMID: 27281280.
- NL Jorstad et al., “Stimulation of functional neuronal regeneration from Müller glia in adult mice,” Nature, 548, 103 (2017). PMID: 28746305.
- D Sanges et al., “Reprogramming Müller glia via in vivo cell fusion regenerates murine photoreceptors,” J Clin Invest, 126, 3104 (2016). PMID: 27427986.
- E Salero et al., “Adult human RPE can be activated into a multipotent stem cell that produces mesenchymal derivatives,” Cell Stem Cell, 10, 88 (2012). PMID: 22226358.
- X Wei et al., “Regeneration of functional retinal ganglion cells by neuronal identity reprogramming,” bioRxiv [Preprint] (2020). DOI: 10.1101/2020.07.16.203497.
- J Wang et al., “Quick Commitment and Efficient Reprogramming Route of Direct Induction of Retinal Ganglion Cell-like Neurons,” Stem Cell Reports, 15, 1095 (2020). PMID: 33096050.
- M Rocha-Martins et al., “De novo genesis of retinal ganglion cells by targeted expression of KLF4,” Development, 146 (2019). PMID: 31405994.