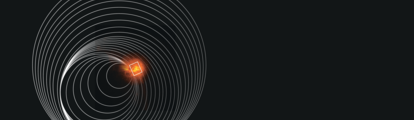
Black Holes and Bright Ideas
When astronomy and ophthalmology collide to propel innovation
In 2007, Damien Gatinel had a conversation with astronomer Jean-Pierre Rivet about astronomy principles and how they relate to ophthalmology (1). Though a connection between the two disciplines may not be immediately apparent to some, there are similarities. In particular, technologies borne from astronomy have influenced the development of tools and techniques that have had a game-changing impact on the diagnosis and treatment of ocular diseases. Rapid advances have occurred since that conversation more than a decade ago, so we felt that it was an ideal time to re-examine the links – and emphasize ways in which one discipline can learn from the other to drive change and innovation.
Where it all began
Original theories linking astronomy and ophthalmology can be traced back to antiquity and the Middle Ages, when the incremental understanding of optics led Ptolemy, Euclid, and Ibn al-Haytham to first propose hypotheses on refraction and the trajectory of light. The Renaissance era further strengthened this relationship, when important research studies, such as those of Kepler, demonstrated direct applications of optics to astronomy and the formed the idea that the image generated on the retina was an inverted one. The works of Descartes, Newton, Snellen, and Fermat gave rise to the modern principles of reflection and refraction, while our contemporary view of light as a wave was described by Christiaan Huygens, and later demonstrated experimentally by Thomas Young in his double slit experiment.
With each era came a deeper understanding of optical systems, our perception of starlight, and the inner workings of the eye. Since ophthalmology and astronomy both rely on the direction and movement of light, the overlap between the two scientific disciplines began to make sense.
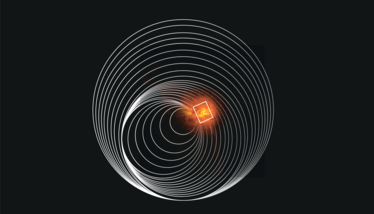
Credit for the central part of the image: NASA/CXC/Villanova University/J. Neilsen.
The eye is a window to the universe
In fact, the eye influenced the development of the first telescope – you may have noticed the striking resemblance between the two. The eye actually served as a major astronomical instrument right up until the invention of the telescope in the 17th century.
Just as the cornea and crystalline lens focus light onto the photoreceptors of the retina, which absorb photons and converts them into a neural impulse, a (modern) telescope uses a sequence of lens elements that focus light from distant sources into a detector, which absorbs photons and ejects electrons – the electrical signal. Unlike the eye, differences in materials and electronics make telescopes sensitive to various wavelengths, from gamma rays to radio.
Two beams, two gamechangers
Moving to the present day, if you take a look at some of the devices that are commonly used in an ophthalmic practice, there are some great examples of astronomy’s influence.
The interferometer is an instrument used to probe distances and make measurements of tiny astronomical objects. In most basic terms, the Michelson interferometer splits laser light into two beams, each traveling different distances before recombining and being recorded by a camera. The instrument leverages the wave-like nature of light; the two beams constructively or destructively interfere, depending on how far one traveled compared with the other. Is this beginning to sound familiar?
Based on these principles, optical coherence tomography (OCT) has today become a standard of care in ophthalmology, providing real-time information on structure and function – diagnosing disease, evaluating progression, and assessing response to therapy. Like interferometry, it’s an instrument that uses two beams of light: one which travels a pre-set distance, while the other varies based on the distance to a patient’s eye. The light reflects off tissue at different depths within the retina, allowing its structure to be probed. In monitoring the progression of glaucoma, for example, an ophthalmologist might use OCT to resolve the layers of the retina to micron-level detail, and identify subtle thinning of the retinal nerve fiber layer (RNFL). It is also commonly used to identify neovascularization and other vascular diseases of the retina, and is increasingly being used to better visualize anterior segment structures (2).
Interferometry principles work well for ophthalmology applications because the distances probed by the former are comparable to the wavelength of light employed by the latter. For example, OCT primarily relies on optical light (in the range of 400-700 nm). Given properties of wave interference and coherence of the light source, this allows ophthalmologists to resolve layers of the retina (for example, the RNFL is approximately 90 µm thick), and structures as small as ∼10 µm in conventional OCT and ∼5 µm in confocal OCT (3, 4). Time-Domain (TD-) OCT uses a moving mirror for the reference beam, whereas more modern Fourier Domain (FD-) or Swept-Source (SS-) OCT eliminate the moving mirror in favor of spectral decomposition.
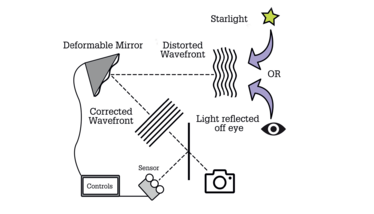
Adaptive Optics System.
Nobel-worthy discoveries
The importance of this approach cannot be understated to astronomers. On 14 September 2015, the microscopic distances probed by interferometry were responsible for revealing signs of a black hole merger (5), marking the first-ever direct observation of gravitational waves – a component of Albert Einstein’s theory of relativity – efforts to prove the existence of which had been ongoing for decades. The discovery was made using the Laser Interferometer Gravitational-Wave Observatory (LIGO), which, at the time, was the largest and most ambitious project ever funded by the National Science Foundation (NSF) in the USA. The LIGO detector consists of two arms – each 4 km in length – along which a laser beam travels before recombining (through a special design the beams are reflected hundreds of times along these arms before recombining, giving LIGO an effective length of ∼1,600 km).
Although the scale of LIGO is markedly different to that of an ophthalmic OCT, surprisingly, its 4 km arms enable even higher precision measurements than those required for ophthalmology. The merger event involving the two black holes, each 30 times the mass of the Sun, distorted space-time via compression along one axis and expansion along the other, oscillating in time. This multiplicative effect changes lengths of one part by 1022, or, in the case of a LIGO arm, ∼10−18 m. Assuming laser light in standard OCT travels a slightly longer distance than the typical axial length, LIGO is analogous to a TD-OCT machine, but 700,000 times larger in size, and in which distortions in space-time are responsible for moving the reference mirror.
Such was the importance of the LIGO detection of gravitational waves, it was awarded the 2017 Nobel Prize in Physics.
Seeking visual clarity
Another parallel between ophthalmology and astronomy can be drawn from a technique that is used to enhance the spatial resolution of images.
Let’s examine the theory. Ideally, the resolution of an optical system is diffraction limited and governed by the Rayleigh Criterion: θ ≈ 1.22 λ/D. Using this formula, optical systems with larger apertures (D) imaging at shorter wavelengths of light (λ) can resolve finer structures. So for reference, the human eye can resolve about one arc-minute, or one-sixtieth of a degree (that’s the apparent size of Jupiter in the sky, which one can see as a disk rather than a point); telescopes can resolve galaxies about 100-1,000 times smaller; and the Event Horizon Telescope, with an effective aperture the size of the Earth, can image a faraway black hole 1,000,000 times smaller (6). Astronomical cameras are matched to telescopes accordingly so that each pixel covers one-half of the size of the smallest resolvable structure (this is called Nyquist sampling). More pixels would not resolve any more detail, and fewer pixels would degrade the resolution.
The human foveola is optimized for the Rayleigh criterion: cones are spaced ∼3 µm apart, or about 0.5 arc-minutes of the eye’s field of view (7). In practice, however, optical images from telescopes on Earth incur blurriness from factors such as turbulence, temperature fluctuations, and water vapor in the Earth’s atmosphere, which distort the incoming wavefront and reduce the resolution of the image (wavefronts are sets of light rays emanating from a source, all at the same phase; they are analogous to the concentric circles of ripples after dropping a pebble in water).
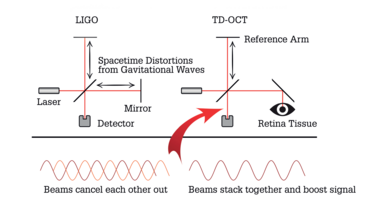
Applications of Michelson Interferometer.
Adapting to adaptive optics
To counter this blurring effect, most modern observatories feature adaptive optics that consist of three key components: i) a Shack Hartmann wavefront sensor, which detects aberrations from an ideal point spread function; ii) software that expresses the aberrations in terms of Zernike polynomials; and iii) pistons that deform the telescope mirror in real-time to negate the aberrations, and bring the optical system closer to its diffraction limit (8, 9).
This same technology can be applied to imaging the eye at high spatial resolution. Akin to the flaws observed with telescopic images of Earth, light reflecting off the eye is subject to imperfections in the eye’s optical train and its various transparent media, and these collectively produce wavefront distortions that limit the finest resolvable detail. By applying adaptive optics, wavefront sensors can be employed to determine the aberration in the eye, while a series of deformable mirrors correct the aberrations and sharpen the resulting image. It should come as no surprise then that adaptive optics are playing an increasingly important role in both anterior and posterior segment imaging and surgery.
Advances in intraoperative aberrometry, for example, have been guided by these principles, allowing surgeons to more accurately identify and correct aberrations, resulting – for most cases – in better postoperative visual outcomes. These new ophthalmic applications may, however, warrant a different mathematical framework, since Zernike polynomials are a mathematical tool commonly used for describing and decomposing distortions to a flat disk only (such as spherical aberration, astigmatism, piston, tilt, tip, defocus, trefoil, coma). But in reality, high-order aberrations (HOAs) – like spherical aberration – also contain the shape and information of low-order ones, too, like defocus, which makes it more difficult to determine clinically significant aberrations. A recently proposed decomposition that treats low- and high-order aberrations separately – but takes both modes into account – may, however, provide a good assessment of the impact of both to allow for more accurate spectacle refraction (10).
Shifting our attention to the posterior segment, adaptive optics also offer many possibilities for improving the resolution of retinal imaging. Most existing standard retinal imaging modalities do not have the resolving power to visualize individual photoreceptors. But the recent integration of adaptive optics modules into existing imaging platforms – such as OCT or scanning laser ophthalmoscopy – now make it possible to view much higher resolution images of microscopic structures in the retina (11). By measuring and “counteracting” the aberrations in light that would normally reduce resolution in the existing imaging platform, adaptive optics-integrated imaging modalities allow for practically direct visualization of foveal rod and cone photoreceptors (12), the retinal pigment epithelium (13), the bodies of retinal ganglion cells (14), and red blood cells (15). Since its capabilities also include indirect visualization of blood flow through retinal vessels, adaptive optics also has the potential to be a groundbreaking tool in monitoring retinal vascular disease (16, 17).
We are optimistic that, as ophthalmic advanced optics continues to evolve, we will be in an even better position to identify inherited retinal diseases – where RPE cells and photoreceptors are the predominantly affected cell types – even before functional changes in vision occur (18)!
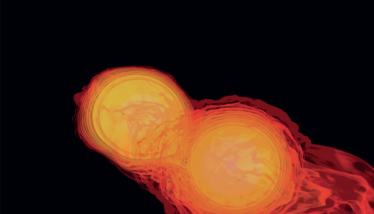
Image credit: NASA’s Goddard Space Flight Center.
Last but not least
We’ve discussed some of the techniques that have had a big impact on our understanding of, and innovation in, ophthalmology and astronomy, but there are others worth mentioning.
Earlier we mentioned FD-OCT – a technique based on interferometry principles – but there are also distinct parallels that can be drawn between the way that FD-OCT separates light to generate ocular structural information, and the way that astronomers analyze the physical properties of a target. To clarify, as well as relying on interferometry, FD-OCT is also reliant on the separation of light into its component wavelengths, whereby a broadband light source is separated by a diffraction grating into its component wavelengths, and distributed across a detector. The relative strengths of each wavelength of light (called a spectrum) encode the structural depth information of the OCT-A-scan. Keeping these principles in mind, in astronomy, a spectrum can help generate all sorts of important physical information, like how hot a star is, how fast a galaxy is moving away from us, whether there is water vapor in the atmosphere of another planet. The importance of this information has made diffraction gratings common components of instruments at many prominent observatories.
And finally, if we consider some of the computational analysis tools that are seeing a surge in interest today, those that are using large image databases for pattern matching and object classification are making a huge difference to both ophthalmologists and astronomers. In ophthalmology, we have witnessed an explosion in the use of machine learning algorithms, such as convolutional neural networks to detect and grade retinal diseases, like diabetic retinopathy. Astronomers use similar algorithms to find gravitational lensing events, whereby a massive object distorts the image of another, in sequences of photographs covering large portions of the sky, or for classifying signals (for example, supernovae, binary stars, luminous galaxies) in time-series data.
In both fields, scientists have turned to the public in making sense of these large datasets, for example, in the 2019 APTOS Blindness Detection (19) and 2018 PLAsTiCC Astronomical Classification challenges (20). These are great examples of multidisciplinary-led innovation.
Onwards and upwards
Our discussion has illustrated some of the key parallels between two distinctly different fields of science and how the evolution of both have guided the understanding of, and innovation within, the other. If we consider some basic facts, the link between ophthalmology and astronomy becomes much clearer. Both use different properties of light and its interaction with matter: its particle nature and the photoelectric effect for imaging sensors; its wave nature for interference patterns; ray tracing for optical system design; and Huygens wavelet theory for wavefront analysis. Despite the substantial differences in the scale of the matter with which they engage, the similarities between the ophthalmology and astronomy demonstrate the importance of interdisciplinary approaches to academic inquiry and how the overlap between fields truly informs growth and drives innovation.
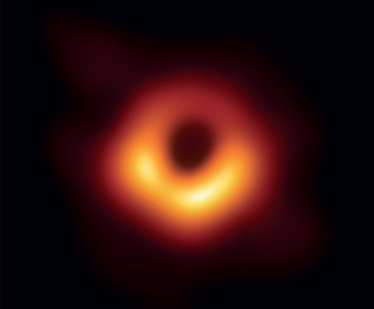
Image credit: European Southern Observatory (ESO).
- D Gatinel and JP Rivet, “Ophtalmologie et astronomie: contrastes et similitudes”, Réalités Ophtalmologiques, 138 (2007).
- JS Asam et al., “Anterior segment OCT”, High Resolution Imaging in Microscopy and Ophthalmology, Cham: Springer International Publishing, 285 (2019).
- DP Popescu et al., “Optical coherence tomography: fundamental principles, instrumental designs and biomedical applications”, Biophys Rev, 3, 155 (2011). PMID: 28510064.
- T Alasil et al., “Analysis of normal retinal nerve fiber layer thickness by age, sex, and race using spectral domain optical coherence tomography”, J Glaucoma, 22, 532 (2013). PMID: 22549477.
- BP Abbott et al., “Observation of gravitational waves from a binary black hole merger”, Phys Rev Lett, 116, 061102 (2016). PMID: 26918975.
- Event Horizon Telescope Collaboration et al., “First M87 event horizon telescope results. I. the shadow of the supermassive black hole”, Astrophysical Journal Letters, 875, L1 (2019).
- J Hirsch and CA Curcio, “The spatial resolution capacity of human foveal retina”, Vision Res, 29,1095 (1989). PMID: 2617858.
- JW Hardy, “Adaptive optics for astronomical telescopes”, Oxford University Press (1998).
- PL Wizinowich et al., “The W. M. Keck observatory laser guide star adaptive optics system: overview”, PASP, 118, 297 (2006).
- D Gatinel et al., “Polynomial decomposition method for ocular wavefront analysis”, J Opt Soc Am A Opt Image Sci Vis, 35, 2035 (2018). PMID: 30645293.
- J Tam, “Adaptive optics and its use in inflammatory eye disease”, Multimodal Imaging in Uveitis, Cham: Springer International Publishing, 135 (2018).
- A Dubra et al., “Noninvasive imaging of the human rod photoreceptor mosaic using a confocal adaptive optics scanning ophthalmoscope”, Biomed Opt Express, 2, 1864 (2011). PMID: 21750765.
- JI Morgan et al., “In vivo autofluorescence imaging of the human and macaque retinal pigment epithelial cell mosaic”, Invest Ophthalmol Vis Sci, 50, 1350 (2009). PMID: 18952914.
- MF Chen et al., “Adaptive optics imaging of healthy and abnormal regions of retinal nerve fiber bundles of patients with glaucoma”, Invest Ophthalmol Vis Sci, 56, 674 (2015). PMID: 25574048.
- P Bedggood and A Metha, “Direct visualization and characterization of erythrocyte flow in human retinal capillaries”, Biomed Opt Express, 3, 3264 (2012). PMID: 23243576.
- B Gu et al., “Noninvasive in vivo characterization of erythrocyte motion in human retinal capillaries using high-speed adaptive optics near-confocal imaging” Biomed Opt Express, 9, 3653 (2018). PMID: 30338146.
- CMA Palochak et al., “Retinal blood velocity and flow in early diabetes and diabetic retinopathy using adaptive optics scanning laser ophthalmoscopy”, J Clin Med, 8 (2019). PMID: 31382617.
- JS Gill et al., “Cellular imaging of inherited retinal diseases using adaptive optics”, Eye (Lond), 33, 1683 (2019). PMID: 31164730.
- Kaggle, “APTOS 2019 blindness detection”, 2019.
- The PLAsTiCC team et al., “The Photometric LSST Astronomical Time-series Classification Challenge (PLAsTiCC): Data set”, arXiv:1810.00001 (2018). Available at: arxiv.org/abs/1810.00001