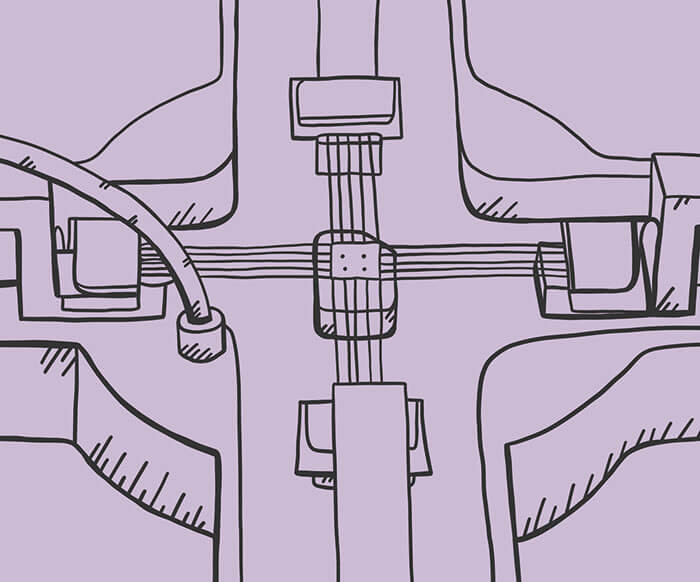
When it comes to rheology – the branch of physics that deals with the deformation and flow of matter, especially the non-Newtonian flow of liquids and the plastic flow of solids – the eye is one hell of a playground. Some structures are somewhat rigid (like the sclera) and others barely at all (the aqueous humor). It’s a pressurized system, drainage issues can cause huge problems, there’s a multitude of muscles that can change not only the direction of the eye at any given moment but also the shape of the tissues inside it. Aging progressively stiffens the principal component of the eye’s focusing system: the lens, and this is all before we get to refractive surgery like astigmatic keratotomy, PRK, LASIK, and SMILE weakening the cornea, let alone any disease states. The cornea is an exquisite example of a close structure-function relationship. It is mechanically strong – strong enough to cope with a wide range of intraocular pressures that can be present in the eye (not just ocular hypertension or glaucoma, but intraocular surgical procedures like cataract surgery, too) and still maintain its geometry. In all of these situations (unless a pathology is present), it is also able to remain transparent throughout life, which is important as the cornea provides about two-thirds of the refractive power of the eye. So the cornea has two main functions: protect the eye and refract light. But even a small change to the structure of cornea can make a big difference to one – or both. The classic example is keratoconus: cone development and progression can rapidly lead to huge dioptric changes in patients’ refraction (and if untreated, ultimately rupture). Further, small arcuate incisions or the laser ablation of relatively small amounts of tissue can both lead to big changes in how the cornea refracts incoming light.
“We’ve known for many years that the topography of a cornea clearly influences its behavior – and many devices have been developed that measure this and track these changes over time,” says University College London’s John Marshall. “It’s allowed us to make assumptions regarding the state of the cornea, and make diagnostic decisions based on them. It is useful information, but it can’t quantify biomechanical properties like corneal stiffness.”
Today’s corneal biomechanical assessments
Peng Shao and Amira Eltony of Harvard Medical School and the Wellman Center for Photomedicine explain traditional limitations. “What we do know about corneal biomechanics has mostly come from ex vivo cornea experiments. Strip extensometry (where the cornea is cut into strips and subjected to uni-axial or bi-axial loads) or pressure inflation experiments have given the vision science community some great insights,” notes Shao. Eltony explained that “You have to bear in mind that these are all destructive tests. They compromise the structural integrity of the cornea where the collagen fibers have been cut, and are limited to the experimental setting.” John Marshall adds that the loading method bears little relationship to physiological loading and that, in the eye, the cornea is curved, which leads to “non-uniform stress distributions.” Given the importance of the cornea to vision, there’s no hope of obtaining biopsy tissue here. A cornea stripped from its pressurized, tensioned native environment can only reveal so much information... Unlike corneal topography, for in vivo, nondestructive assessments of corneal biomechanics, Marshall notes that “you currently need dynamic measurements to quantify biomechanical properties at any given time, like tracking the change of shape of the cornea in response to a measured load” – just like those provided by Reichert’s Ocular Response Analyser (ORA) and Oculus’ Corvis Dynamic Scheimpflug Tonometer (ST). Both use a puff of air to deflect the cornea. ORA uses an infrared beam and both use very high-speed cameras to track how the cornea responds to this deflection, capturing the ripples out to the periphery. Appropriate processing of the ORA infrared waveforms that return can give you some useful parameters – corneal hysteresis (CH; the ability of the cornea to absorb and dissipate the energy from the air puff – in other words, the rate-dependent viscoelastic response), corneal resistance factor (CRF; the total visco-elastic response of the cornea) and “corneal compensated” IOP (IOPCC). This last one is important – IOP measurements using the trusty old Goldman tonometer have long been known to be affected by corneal thickness and stiffness – and topical prostaglandin therapies are known to soften the cornea, so it’s valuable to be able to measure IOP without the influence of these confounding factors. With the Corvis ST, the depth of air puff-induced deformation can be measured and, once IOP has been taken into account, should be primarily related to corneal biomechanical properties. But again, there are limitations with these methods. The data are only gathered from the center of the cornea and under pressures that aren’t physiological in terms of either magnitude or direction. Further, CH, CRF, and other deformation parameters have been shown to be influenced by other factors, such as differences in central corneal thickness and IOP (1). Julian Stevens, Consultant Ophthalmologist at London’s Moorfields Eye Hospital puts it this way, “With these techniques, we’re essentially bouncing the cornea like a trampoline. The reality is, measuring IOP is important, but actually, once you know how stiff the sclera and cornea are, then it becomes a much more interesting number than just the headline IOP on its own, and the individual importance of the IOP can be much better understood.” There are a number of other approaches, such as Placido disk imaging or optical coherence elastography, which can be used to measure corneal shape changes after corneal indentation by interventions like a puff of air or a concave lens. But as Eltony explains, “They all share the same problem: these are at best an overall corneal biomechanical measurement. They can’t detect localized stiffening or weakening in the cornea.” If you want a stiffness map (and if you’re dealing with a patient with prior refractive surgery or a corneal ectatic disorder, you really do), these classic mechanical approaches won’t give you the right information. But two other non-contact approaches look like they can: Brillouin spectroscopy and laser interferometry.The next generation
Brillouin spectroscopy
Brillouin spectroscopy is a quantum mechanical process that isn’t directly comparable to the classic mechanical assessments described above. This approach does not involve any dynamic or shape-changing processes, but probes biomechanical properties of (quantum) mechanical fluctuations on an atomic level (or by its wave analogy). Probing is instead performed non-invasively by a dynamic process: the analysis of photon-phonon interactions.To go any further in this story, we need to understand what a phonon is. Quantum mechanics textbooks would describe a phonon as “the elementary vibrational motion in which a lattice of atoms or molecules uniformly oscillate at a single frequency.” It’s perhaps more helpful to view phonons as a description of the collective excitation of molecules or atoms in condensed matter. In tissues, phonons are present due to the thermodynamic fluctuations of the molecules and atoms that constitute those tissues, and phonons can be also created by light. These spontaneous mechanical fluctuations in the tissue can also be probed by light: photons from the light source enter, interact with these acoustic phonons and scatter the light in a characteristic manner. Brillouin spectroscopy (Figure 1) is the measurement of spectral changes in how light is scattered by an object – and it reveals information on the phonon’s properties, and therefore (and crucially), the viscoelastic properties of the medium. In other words, for each point a scanning confocal laser beam hits, the instrument detects the spectral shift between the outgoing light and the light that returns. This should be directly correlated with the modulus of elasticity at that point, meaning you can map in all three dimensions and generate a stiffness map. The only problem? Other phenomena scatter the light too, and the frequency shifts involved with the Brillouin scattering are in the gigahertz range and have a very faint signal strength. What’s really held Brillouin spectroscopy back for years has been signal detection. When it comes to assessing the biomechanics of an inanimate object, detectors like Fabry–Pérot interferometers or angle-dispersive etalons did the job – as the object is inanimate, it can be imaged for as long as is needed. But for biomedical imaging, these approaches aren’t good enough – they were either too slow, or their signal-to-noise ratio was too low to be useful. A breakthrough came in 2007 at Harvard University and Massachusetts General Hospital when Giuliano Scarcelli and Seok-Hyun (Andy) Yun managed to combine Brillouin spectroscopy and confocal microscopy with a virtually imaged phase array (VIPA) detector that enabled very high throughput and efficient spectral separation. The speed and signal-to-noise ratios were high enough, and multiple frequencies could be detected at the same time, speeding the acquisition time (2). Achievement unlocked. However, the gap between demonstrating proof-of-concept and actually having a product that clinicians can use can be huge. OCT took seventeen years from concept to clinic – and in the case of the Harvard team, a far speedier nine years to develop the technology to a stage where it was fast and sensitive enough for clinical use. The Brillouin confocal microscope is now called the Brillouin Optical Scanner System (BOSS), and is being commercialized by Intelon Optics; a prototype is now being used clinically.
Laser interferometry
The other approach is laser interferometry. It uses the principle that if you can view the displacement of an object in response to a known load, you can determine several useful properties of the material – including stiffness (3,4). Here, displacement is measured by holography – or its digital form, electronic speckle pattern interferometry (ESPI) – and it can be used to create 3D phase-related displacement fields for mapping. John Marshall explains, “A monochromatic coherent laser is split into two: one wavefront illuminates the object, the other acts as a reference beam. Both are combined in an imaging device. The resulting image is a pattern of speckles that encodes information on the wavelength displacement of returning light. In principle, performing laser interferometry is pretty simple: take a reference measurement and take another measurement after applying a load, then subtract the speckle patterns” (Figure 2). A similar technique, electronic speckle pattern shearing interferometry (ESPSI) can also be used to measure the surface strains of a sample after a mechanical load (3,4); rather than using a reference beam, the object is used as its own reference. The wavefront that returns from the object is transformed from the original wavefront and interferes with it.“Shearing interferometry is performed by splitting the wavefront into two parts – one part is transformed by the object in a specific way, and the wavefront is recombined to give a specific speckle interference pattern – and as before, two measurements are made, before and after displacement, followed by a subtraction of both patterns,” says Marshall. “This gives you is the rate of displacement, i.e. strain – or information on which areas of a structure are weaker and which are stronger.” ESPI and ESPSI are widely used in engineering for applications like the detection of cracks in aircraft wings, or vibration and strain monitoring – but could also of considerable utility in understanding the biomechanics of the eye. The development of an in vivo device is currently ongoing.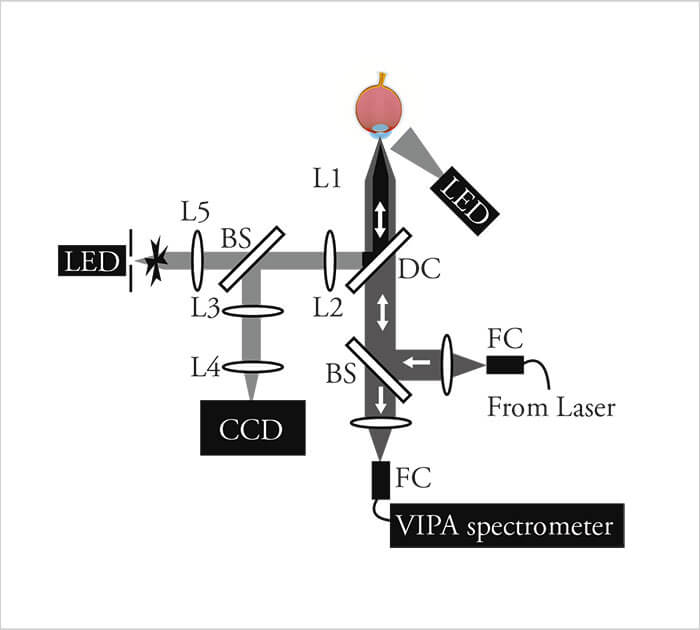
What’s the potential?
Corneal ectasia screening and corneal collagen cross-linking
Let’s start with refractive surgery and with “do no harm.” LASIK-induced ectasia is the stuff of nightmares – that’s why there is a whole spectrum of diagnostic procedures to help avoid causing it, from genetic tests to corneal topography. But forme fruste keratoconus (or any other subclinical weakening of the cornea) is incredibly challenging to detect with corneal topography; it’s suspected or identified based on very subtle changes. But it’s the weakening of corneal collagen fibers that results in the changes in corneal topography (5,6) – and if Brillouin microscopy or the laser interferometry approach can detect the weakening at an earlier stage than other methods, many patients who would have otherwise undergone laser refractive surgery and gone on to develop ectasia would be spared the ordeal. There’s also a more obvious application: optimizing corneal collagen cross-linking (CXL) and monitoring post-CXL corneas for any signs of ectasia progression (7). Before performing CXL, knowledge of the strongest and weakest regions of the cornea (Figure 3) is particularly valuable when it comes to optimizing beam profiles and scanning patterns. But it’s the knowledge of how effective the procedure has been in strengthening a patient’s cornea that will feed into the optimization of not only how the light is delivered, but also which riboflavin solution works best under certain circumstances. Stevens notes, “We need to get a lot smarter with CXL to ensure that each eye that’s cross-linked is properly cross-linked and we need a measurement of strength to reference the individual cornea against the population distribution.” Next generation corneal biomechanical assessments should help with that.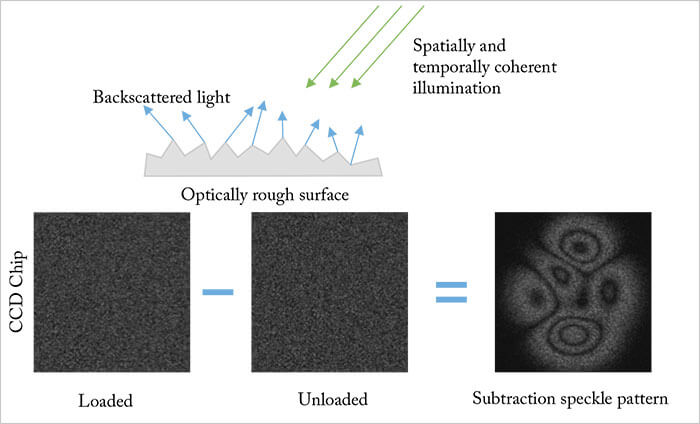
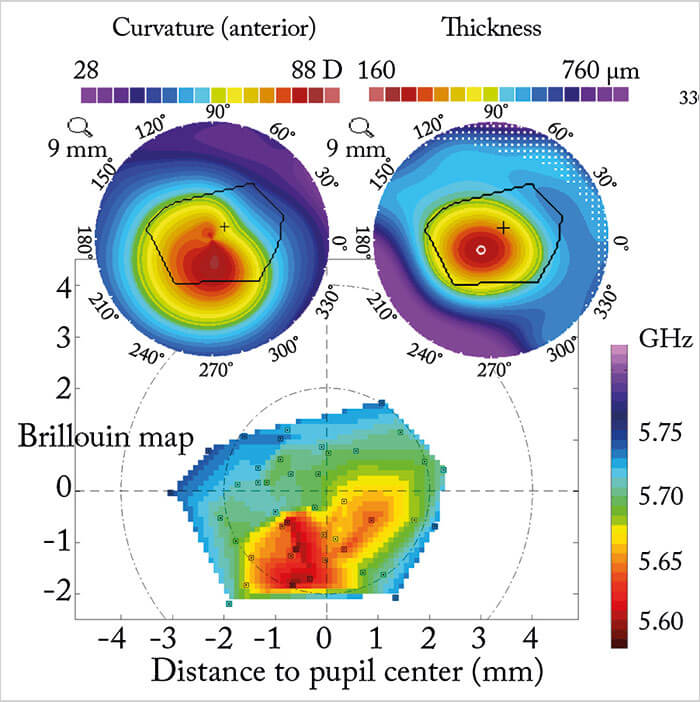
Brillouin microscopy has already been used to measure the differences in corneal elasticity before and after CXL – and to assess novel CXL techniques (Figure 4) (8,9). The corneal flattening effects of CXL are also being investigated for the treatment of low myopia – and it’s clear how knowledge gained through the use of corneal Brillouin microscopy or laser interferometry might help optimize the procedure.
Laser refractive surgery
Every cornea has its own unique biomechanical properties and, when it comes to incisions or ablations, each cornea reacts in a slightly different way – not only to the procedure, but also in recovery. A better understanding of each patient’s individual corneal biomechanics before and after refractive surgery should help further optimize current finite element models of the cornea and how it reacts to surgery – ultimately leading to more accurate outcome predictions. Such knowledge could also predict the amount of surgically induced astigmatism (SIA) that is caused during any procedure that involves corneal incisions (like cataract surgery). It also means you could plan a strategy that would correct for a large proportion of SIA, well in advance of scrubbing up for surgery. “The astigmatism nomograms for astigmatism correction in cataract surgery take into account SIA,” says Stevens. “If you go to a nomogram calculator website like my own (Figure 5), the first thing you have to put in is your own SIA. So with my standard incisions, I get an overall 0.3 DC against-the-wound shift. So with a temporal incision, in those patients who do not undergo astigmatic correction, the overall effect is a 0.3 DC push vertically – so I steepen the vertical meridian by 0.3 DC. That’s my standard across my overall population. But in reality, each eye is different. I have some eyes which have 1.0 DC of shift. Others have zero shift at all. Some of that’s topographic noise, some of that’s any other number of factors, including epithelium, tear film changes and many other factors. But if we had knowledge of the individual cornea’s biomechanics, we’d be able to get about a 50 percent improvement in astigmatic treatment outcomes using intrastromal femtosecond laser arcs.”Glaucoma and ocular hypertension
A better understanding of the biomechanics of the eye has other applications too – like characterizing the stiffness of the trabecular meshwork (TM). One of the hallmarks of primary glaucoma is the accumulation of glycosaminoglycans in the extracellular matrix and a thickening of TM beams (10). This results in a loss of trabecular spaces and, combined with chronic inflammatory changes, appears to alter the biomechanics of the TM; it becomes stiffer, changes outflow and can influence the onset and progression of glaucoma (11,12). If you’re able to measure TM stiffness, it not only helps screen for potential problems, it also opens up a potential new pharmacological approach for glaucoma therapy – as well as a measurable endpoint to test any such therapy’s efficacy (12).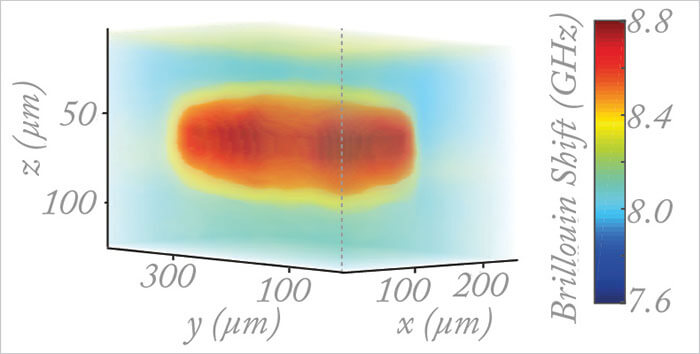
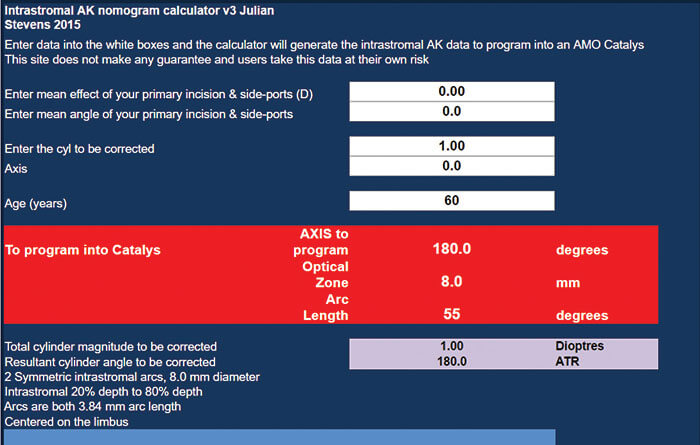
And there’s another application in glaucoma: scleral biomechanics. A number of biomechanical models have suggested that the sclera transmits IOP-induced mechanical strain to the optic nerve head (13), and experiments in ex-vivo human eyes have confirmed that the greatest scleral strain is in the peripapillary region (14). A number of mouse studies have suggested that eyes that are stiffer at baseline (and therefore more resistant to elongation) are less likely to experience one of the defining features of glaucoma: retinal ganglion cell (RGC) loss (15,16). Could peripapillary scleral collagen cross-linking (SXL) help protect eyes with elevated IOP from RGC loss and optic nerve damage? Performing SXL is easier said than done – the sclera can be difficult to access, care needs to be taken to avoid damaging extraocular muscles, and issues that pertain to uniform light delivery need to be resolved – but all of these aspects look like they can be overcome (17). It’s also likely that the exact positioning and amount of SXL needs to be individualized, which is where techniques like Brillouin microscopy or laser interferometry might come in. Stevens notes that “If SXL can be successfully performed, then there’s another potential application: arresting scleral elongation to control myopia and prevention.”
The aging lens
Presbyopia can also be considered a biomechanical problem. It’s widely accepted that the natural crystalline lens gradually loses elasticity as people age, with a subsequent decrease in accommodation range – but the specifics (changes in lens stiffness with age and how much it compromises accommodation) remain unknown. Although the lens can be imaged (and its ability to accommodate) in vivo with ultrasound biomicroscopy, OCT, or even magnetic resonance imaging, not one of those methods reveals anything about lens stiffness. Brillouin microscopy has already been used to show that, in mice, the lens nucleus is considerably stiffer than the cortex, and saw a “marked age-related stiffening” (18). In vivo Brillouin sagittal stiffness profiles have already been characterized in humans – from young adults to those in their seventh decade (Figure 6) (19).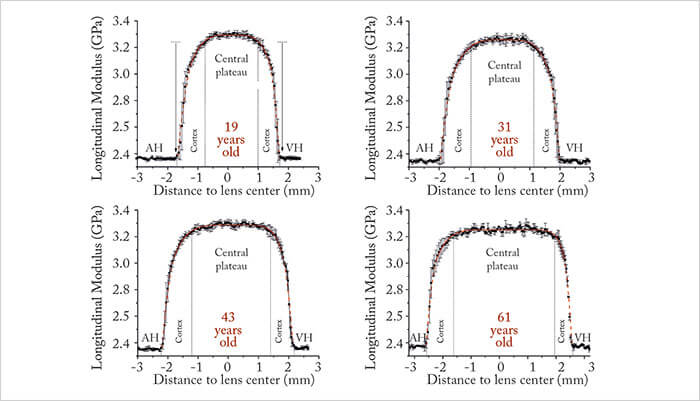
With lens-softening eyedrop treatments for presbyopia on the horizon – be it lanosterol or Novartis’ EV06 compound under clinical evaluation – the role of any technology that can non-invasively assess their impact is not hard to imagine. However, Stevens notes, “The aging lens probably has some other degradations in terms of fibrillation of lens crystalline bundles and fibers, and just general disorganization and damage. So crystalline lens softening will have limits in restoring lens shape change and will not reorganize disordered crystalline lens fibers. But these techniques are the way of measuring lens stiffness in vivo and will be essential to understanding future presbyopia treatments.”
Following in Hubble’s footsteps
A better understanding of in vivo ocular biomechanics has the potential to offer better screening of patients for ocular disease, meaning earlier identification and more timely intervention. It could also mean that refractive surgery can become more personalized, predictable – and with better patient outcomes. And biomechanically-guided SXL could prove to be an effective treatment for glaucoma or for the prevention ofpathologic myopia. Right now, instruments like in vivo interferometers and BOSS are still a number of years away from being commercially available. But if they can be brought into the clinic, imagine the potential benefits it could bring to a whole spectrum of patients faced with a whole spectrum of diseases. Julian Stevens views such technology as “the Hubble space telescope of ophthalmology – you can actually assess ocular biomechanics directly” and believes that it will rapidly change the way ophthalmologists and optometrists think about the cornea. “The scanner on its own provides data – data that will improve in quality over the next 5–10 years. But that data will be integrated into finite element models of the cornea. And very soon, we will have a whole lot of data alongside back-end intelligence to help interpret the scans you perform. It exactly like cardiologists’ ECG scans, which come with very sophisticated deep learning AI analysis. Soon, we’ll have the same for the biomechanics of the eye. We’re going to get new insights and improve what we do. It’s as simple as that.” John Marshall reports no commercial interests in the technology and products mentioned in this article. Julian Stevens reports that he is a consultant to Intelon Optics, STAAR Surgical AG, Abbott, VistaOptical, Oculentis AG and Revision Optics. Peng Shao and Amira Eltony are Harvard Medical School research fellows under the supervision of Andy Yun, Scientific Founder and board member of Intelon Optics
References
- M Matsuura et al., “The usefulness of CorvisST Tonometry and the Ocular Response Analyzer to assess the progression of glaucoma”, Sci Rep, 7, 40798 (2017). PMID: 28094315. G Scarcelli, SH Yun, “Confocal Brillouin microscopy for three-dimensional mechanical imaging”, Nat Photonics, 2, 39–43 (2007). PMID:19812712 A Wilson, J Tyler, J Marshall, “3. Laser interferometry”, in CJ Roberts, J Lia (Eds), “Corneal Biomechanics: From Theory to Practice, 1st Edition”. Kugler Publications Amsterdam (2016). A Wilson, J Marshall, JR Tyrer, “The role of light in measuring ocular biomechanics”, Eye (Lond), 30, 234–240 (2016). PMID: 26768916. MK Smolek, “Interlamellar cohesive strength in the vertical meridian of human eye bank corneas”, Invest Ophthalmol Vis Sci, 34, 2962–2969 (1993) PMID: 8360028. JB Randleman et al., “Depth-dependent cohesive tensile strength in human donor corneas: implications for refractive surgery”, J Refract Surg, 24, S85–89 (2008). PMID: 18269156. MJ Girard et al. “Translating ocular biomechanics into clinical practice: current state and future prospects”, Curr Eye Res, 40, 1–18 (2015). PMID: 24832392. G Scarcelli et al., “Brillouin microscopy of collagen crosslinking: noncontact depth-dependent analysis of corneal elastic modulus”, Invest Ophthalmol Vis Sci, 54, 1418–1425 (2013). PMID: 23361513. SJJ Kwok et al., “Selective two-photon collagen crosslinking in situ measured by Brillouin microscopy”, Optica, 3, 469–472 (2016). PMID: 25611213. Available at: bit.ly/2lyJc2Q. R Sihota et al., “Scanning electron microscopy of the trabecular meshwork: understanding the pathogenesis of primary angle closure glaucoma”, Indian J Ophthalmol, 60, 183–138 (2013). PMID: 22569378. JA Last et al., “Elastic modulus determination of normal and glaucomatous human trabecular meshwork”, Invest Ophthalmol Vis Sci, 52, 2147–2152 (2011). PMID: 21220561 CT McKee et al., “The effect of biophysical attributes of the ocular trabecular meshwork associated with glaucoma on the cell response to therapeutic agents”, Biomaterials, 32, 2417–2423 (2011). PMID: 21220171. HA Quigley, EM Addicks, “Regional differences in the structure of the lamina cribrosa and their relation to glaucomatous optic nerve damage”, Arch Ophthalmol, 99, 137–143 (1981). PMID: 7458737. MA Fazio et al. “Regional variations in mechanical strain in the posterior human sclera”, Invest Ophthalmol Vis Sci, 53, 5326–5333 (2012). PMID: 22700704. FE Cone et al., “Differential susceptibility to experimental glaucoma among 3 mouse strains using bead and viscoelastic injection”, Exp Eye Res, 91, 415–424 (2010). PMID: 20599961. MR Steinhart et al., “Mice with an induced mutation in collagen 8A2 develop larger eyes and are resistant to retinal ganglion cell damage in an experimental glaucoma model”, Mol Vis, 18, 1093–1106 (2012). PMID: 22701298. M Kim et al., “Flexible elastomer optical waveguide for whole-glove scleral crosslinking”, Presented at the International CXL Experts Meeting, December 3rd, 2016; Zurich, Switzerland. Available at: bit.ly/2msWTnX. G Scarcelli, P Kim, SH Yun, “In vivo measurement of age-related stiffening in the crystalline lens by Brillouin optical microscopy”, Biophys J, 101, 1539–1545 (2011). PMID: 21943436. S Besner et al., “In Vivo Brillouin Analysis of the Aging Crystalline Lens”, Invest Ophthalmol Vis Sci, 57, 5093–5100 (2016). PMID: 27699407.